The BPSC General Studies Manual is designed to cover the syllabus of Prelims and Mains Examination conducted by BPSC (Bihar Public Service Commission). Few pages from the e-Book:
Continental Drift
Continental drift is a scientific theory that was developed in the early 20th century by German meteorologist and geophysicist Alfred Wegener. It proposed that Earth’s continents were once part of a single, supercontinent called Pangaea and have since drifted apart to their current positions. Continental drift is a key concept in the theory of plate tectonics, which is now widely accepted in the field of geology.
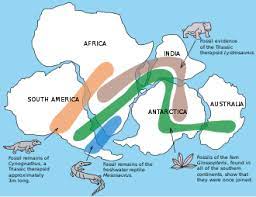
Key Points of Continental Drift:
- Pangaea: Wegener’s theory postulated the existence of Pangaea, a supercontinent that existed approximately 335 to 175 million years ago during the late Paleozoic and early Mesozoic eras. Pangaea means “all lands” in Greek.
- Evidence:
- Fossil Evidence: Wegener observed that identical fossils of plants and animals were found on continents that are now widely separated by oceans. This suggested that these continents were once connected.
- Geological Evidence: Similar rock formations, mountain ranges, and geological structures were found on continents that are now far apart, indicating that they were once joined.
- Paleoclimatic Evidence: Wegener noted that evidence of past glaciations and climatic conditions, such as glacial deposits and coal beds, could be correlated across continents, even though they are now in different latitudinal positions.
- Continental Drift Mechanism: Wegener proposed that the continents “drifted” due to the force of tidal gravitational interactions caused by the Moon and the Sun. However, this mechanism was later discredited.
- Criticism and Acceptance: Initially, Wegener’s theory faced skepticism from the scientific community because he couldn’t provide a convincing mechanism for how continents could move. It wasn’t until the mid-20th century, with the development of the theory of plate tectonics, that the idea gained widespread acceptance.
Seafloor Spreading
Seafloor spreading is a fundamental geological process that occurs at mid-ocean ridges, where new oceanic crust is formed through volcanic activity and then gradually moves away from the ridge in both directions. This process is a key component of the theory of plate tectonics and provides essential insights into the dynamics of the Earth’s lithosphere (the rigid outer layer of the Earth).
Key Points of Seafloor Spreading:
- Mid-Ocean Ridges:
- Seafloor spreading primarily takes place along mid-ocean ridges, which are long underwater mountain chains that run through all major ocean basins. The mid-Atlantic Ridge and the East Pacific Rise are prominent examples.
- These ridges mark the boundaries between tectonic plates.
- Formation of New Oceanic Crust:
- At mid-ocean ridges, the Earth’s lithosphere is being pulled apart due to tectonic forces. As a result, magma (molten rock) from the Earth’s mantle rises to the surface through fractures and fissures created by the separation of tectonic plates.
- When this magma solidifies upon contact with cold seawater, it forms new oceanic crust. This process is called volcanic extrusion.
- Symmetrical Spreading:
- The new oceanic crust is created symmetrically on both sides of the mid-ocean ridge. As magma continues to well up, it pushes the existing crust aside, causing the oceanic plates to move away from the ridge axis.
- This creates a pattern of symmetry, with older and colder oceanic crust farther away from the ridge and younger and hotter crust near the ridge axis.
- Recording Magnetic Reversals:
- One of the key pieces of evidence for seafloor spreading comes from the study of magnetic anomalies on the seafloor. As new oceanic crust forms, it inherits the Earth’s magnetic field orientation at the time of its solidification.
- The Earth’s magnetic field periodically reverses its polarity, causing magnetic north to become magnetic south and vice versa. These magnetic reversals leave a record in the rocks.
- By studying the magnetic properties of seafloor rocks, scientists have identified alternating magnetic stripes of normal and reversed polarity parallel to mid-ocean ridges. These stripes are symmetrical on either side of the ridge and provide strong evidence for seafloor spreading.
- Rate of Seafloor Spreading:
- The rate of seafloor spreading varies from one location to another but is typically a few centimeters per year. This movement contributes to the overall movement of tectonic plates on the Earth’s surface.
- Consequences:
- Seafloor spreading is linked to other geological processes, such as subduction at convergent plate boundaries, where older oceanic crust sinks beneath continental or other oceanic plates. This process helps to recycle the Earth’s lithosphere.
In summary, seafloor spreading is the process by which new oceanic crust is formed at mid-ocean ridges, leading to the gradual widening of ocean basins and the movement of tectonic plates. The evidence for seafloor spreading includes the symmetrical magnetic striping of the seafloor, which supports the theory of plate tectonics and our understanding of the dynamic nature of the Earth’s lithosphere.
Movement of Plates
The movement of tectonic plates is a fundamental geological process that shapes the Earth’s surface and drives various geological phenomena, such as earthquakes, volcanic eruptions, and the formation of mountains and ocean basins. This movement is a central concept in the theory of plate tectonics, which is a unifying theory in the field of geology.
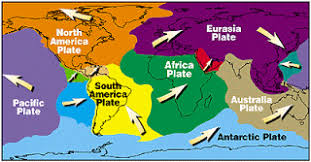
Key Points of Plate Movement:
- Tectonic Plates: The Earth’s lithosphere (the rigid outer layer) is divided into several large and numerous small tectonic plates. These plates are composed of the Earth’s crust and the uppermost part of the mantle.
- Plate Boundaries: Plate movement primarily occurs at plate boundaries, which are the boundaries where two tectonic plates interact. There are three main types of plate boundaries:
- Divergent Boundaries: Plates move away from each other. This can lead to the formation of new oceanic crust as magma rises to the surface, creating mid-ocean ridges and rift valleys.
- Convergent Boundaries: Plates move toward each other. This can result in plate collision, subduction (one plate diving beneath another), and the formation of mountain ranges, deep-sea trenches, and volcanic arcs.
- Transform Boundaries: Plates slide past each other horizontally. This lateral movement can cause earthquakes along fault lines.
- Driving Forces:
- Plate movement is primarily driven by the convection currents in the Earth’s mantle, which is the semi-fluid layer beneath the lithosphere. These convection currents are generated by the heat produced by the decay of radioactive isotopes in the Earth’s interior.
- As heated mantle material rises toward the Earth’s surface, it pushes the overlying tectonic plates away, creating divergent boundaries. Conversely, as cooled material sinks back into the mantle, it can pull plates with it, creating convergent and transform boundaries.
- Rate of Plate Movement: The rate of plate movement varies but is generally slow, typically on the order of a few centimeters per year. This movement is continuous and relentless, accumulating over geological time.
- Consequences of Plate Movement: Plate movement is responsible for a wide range of geological phenomena:
- Earthquakes: These occur along fault lines, particularly at transform boundaries, when the accumulated stress is suddenly released.
- Volcanic Activity: Volcanic eruptions are common at convergent boundaries (where one plate is forced beneath another) and divergent boundaries (where new oceanic crust is formed).
- Mountain Building: The collision of continental plates at convergent boundaries can lead to the formation of mountain ranges.
- Ocean Basin Formation: The spreading apart of plates at mid-ocean ridges creates new oceanic crust and leads to the expansion of ocean basins.
- Recycling of the Lithosphere: Plate movement is part of a cycle that recycles the Earth’s lithosphere. At convergent boundaries, older oceanic crust may be subducted, melted, and recycled into the mantle.
In summary, the movement of tectonic plates is driven by the convection currents in the Earth’s mantle and occurs primarily at plate boundaries. This movement shapes the Earth’s surface, causes geological phenomena, and plays a crucial role in the dynamic processes that have shaped our planet’s geological history.
Earthquakes
An earthquake is a sudden and violent shaking of the Earth’s surface, often caused by the release of energy stored in the Earth’s crust due to geological processes. Earthquakes can result in the ground shaking, causing damage to structures, landforms, and sometimes even loss of life.
Causes of Earthquakes: Earthquakes are primarily caused by the movement of the Earth’s tectonic plates, which make up the Earth’s outer shell. There are three main types of plate boundaries where most earthquakes occur:
- Transform Boundaries: At transform boundaries, tectonic plates slide past each other horizontally. The friction between the plates prevents them from moving smoothly, causing stress to build up. When the stress is released suddenly, it results in an earthquake.
- Convergent Boundaries: At convergent boundaries, tectonic plates move toward each other. When two plates collide or one plate subducts beneath another, immense pressure can build up, leading to an earthquake when the pressure is released.
- Divergent Boundaries: At divergent boundaries, tectonic plates move away from each other. As plates separate, magma from the mantle can rise to fill the gap, creating tensional stress that can lead to earthquakes.
Seismic Waves: When an earthquake occurs, it generates seismic waves that radiate outwards from the earthquake’s epicenter, which is the point on the Earth’s surface directly above the earthquake’s origin (the focus or hypocenter). There are two main types of seismic waves:
Body Waves: These waves travel through the Earth’s interior and include two types:
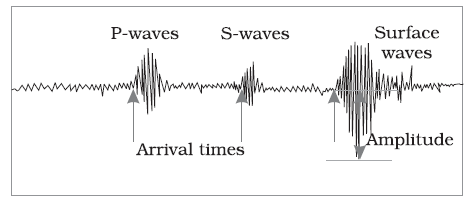
Primary (P) Waves: P-waves are compressional waves that travel through solids, liquids, and gases. They are the fastest seismic waves and are responsible for the initial, less-damaging shaking during an earthquake.
Secondary (S) Waves: S-waves are shear waves that can only travel through solids. They are slower than P-waves and cause more intense shaking, often responsible for structural damage.
Surface Waves: Surface waves travel along the Earth’s surface and include two types:
-
- Love Waves: Love waves cause horizontal shearing motion and can damage buildings and infrastructure.
- Rayleigh Waves: Rayleigh waves have rolling, up-and-down motion and can cause significant ground displacement.
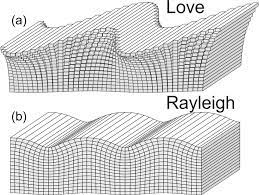
Measuring Earthquakes: The magnitude of an earthquake is commonly measured using the Richter scale or the moment magnitude scale (Mw). These scales quantify the energy released by an earthquake and provide a single numerical value to represent its size. The Mercalli Intensity Scale, on the other hand, measures the intensity or the effects of an earthquake at specific locations.
Impact and Mitigation:
- Earthquakes can have devastating consequences, including building collapse, landslides, tsunamis (if they occur under the ocean), and loss of life.
- Preparedness, early warning systems, and construction practices that consider seismic risk are essential for mitigating the impact of earthquakes.
- Seismologists study earthquakes to better understand their causes and effects, which can lead to improved prediction and preparedness efforts.
In summary, earthquakes are natural geological phenomena caused by the movement of tectonic plates. They generate seismic waves that can lead to ground shaking and various types of damage. Understanding the causes and effects of earthquakes is crucial for minimizing their impact and ensuring the safety of communities in earthquake-prone regions.
How The Earthquakes Occur?
Earthquakes occur due to the sudden release of energy within the Earth’s crust, resulting in the shaking or movement of the Earth’s surface. This release of energy is primarily caused by the movement of tectonic plates, which make up the Earth’s outer shell. Here’s a more detailed explanation of how earthquakes occur:
- Tectonic Plate Movement: The Earth’s lithosphere is divided into several large and small tectonic plates that float on the semi-fluid asthenosphere beneath them. These plates are constantly in motion, although their movements are generally slow, typically a few centimeters per year. There are three main types of plate boundaries where tectonic plates interact:
- Convergent Boundaries: At convergent boundaries, tectonic plates move toward each other. When two plates collide, the immense pressure caused by the plates’ compression can lead to the accumulation of stress and the potential for earthquakes.
- Divergent Boundaries: At divergent boundaries, tectonic plates move away from each other. As plates separate, magma from the mantle can rise to fill the gap, creating tensional stress that can lead to earthquakes.
- Transform Boundaries: At transform boundaries, tectonic plates slide past each other horizontally. The friction between the plates prevents them from moving smoothly, causing stress to build up. When the stress is released suddenly, it results in an earthquake.
- Stress Accumulation: As tectonic plates interact at these boundaries, they can become locked together due to friction. This locking prevents the plates from freely moving, resulting in the accumulation of stress along fault lines. Stress can continue to build up for years or even centuries.
- Release of Energy: Eventually, the accumulated stress reaches a critical point where the friction can no longer hold the plates together. When this happens, the plates suddenly slip or rupture along a fault line. This sudden release of stored energy generates seismic waves that radiate outwards from the point of rupture.
- Seismic Waves: Seismic waves are the energy waves produced by the earthquake. There are two main types of seismic waves:
- Primary (P) Waves: P-waves are compressional waves that travel through solids, liquids, and gases. They are the fastest seismic waves and arrive at seismometers first.
- Secondary (S) Waves: S-waves are shear waves that can only travel through solids. They are slower than P-waves and cause more intense shaking.
- Surface Shaking: As seismic waves reach the Earth’s surface, they cause the ground to shake, resulting in the felt effects of an earthquake. The severity of the shaking depends on factors such as the earthquake’s depth, magnitude, and distance from the epicenter.
- Aftershocks: After the main earthquake, there can be a series of smaller earthquakes known as aftershocks. These aftershocks are caused by continued adjustments in the Earth’s crust following the initial rupture.
In summary, earthquakes occur due to the movement of tectonic plates and the release of stress that has built up along fault lines. This release of energy generates seismic waves that result in ground shaking. Understanding the causes and effects of earthquakes is crucial for earthquake preparedness and mitigating their impact on communities in earthquake-prone regions.
Determination of Epicentre
The epicenter of an earthquake is the point on the Earth’s surface directly above the earthquake’s origin or focus (hypocenter), where the seismic waves generated by the earthquake first reach the surface. Determining the epicenter is a critical aspect of earthquake monitoring and understanding its geographical impact. Here’s how the epicenter of an earthquake is determined:
- Seismic Network:
- Earthquakes are monitored globally by a network of seismometers, which are instruments that detect and record ground motion caused by seismic waves.
- These seismometers are strategically placed in various locations around the world, including seismically active regions, to continuously monitor ground movements.
- Seismic Wave Arrival Times:
- When an earthquake occurs, seismic waves are generated at the earthquake’s focus (hypocenter). These waves radiate outward in all directions through the Earth’s interior.
- Recording the Seismic Waves:
- Seismometers detect the arrival of seismic waves at their respective locations. They record the time when the waves arrive and their amplitude (intensity).
- Triangulation:
- To determine the epicenter, seismologists rely on the time differences in the arrival of seismic waves at multiple seismometer stations.
- By comparing the arrival times of seismic waves at three or more different seismometer stations, seismologists can triangulate the earthquake’s epicenter.
- Travel Time Curves:
- Seismologists use travel-time curves or tables that show the expected arrival times of seismic waves at different distances from the earthquake’s epicenter.
- By comparing the actual arrival times recorded by the seismometers with the expected arrival times, seismologists can calculate the distances from each station to the earthquake’s epicenter.
- Intersection Point:
- The intersection point of the calculated distances from the different seismometer stations represents the earthquake’s epicenter.
- The more seismometer stations involved in the calculation, the more accurately the epicenter can be determined.
- Magnitude Determination:
- In addition to determining the epicenter, seismologists also calculate the earthquake’s magnitude, which is a measure of its energy release. The magnitude is determined based on the amplitude of seismic waves recorded at multiple stations.
- Reporting:
- Once the epicenter and magnitude are determined, this information is reported to relevant authorities and agencies responsible for earthquake monitoring and emergency response.
In summary, determining the epicenter of an earthquake involves a process of triangulation using data from multiple seismometer stations. By comparing the arrival times of seismic waves at these stations, seismologists can accurately pinpoint the location on the Earth’s surface directly above the earthquake’s origin. This information is crucial for assessing earthquake hazards and responding to seismic events.
Earthquake Belts
Earthquake belts, also known as seismic belts or earthquake zones, are geographic regions where earthquakes are concentrated due to the tectonic activity occurring beneath the Earth’s surface. These belts are characterized by a higher frequency and intensity of seismic events compared to areas outside the belts. Earthquake belts are primarily associated with the boundaries of tectonic plates and are a result of the interactions between these plates. Here are some of the major earthquake belts around the world:
- Pacific Ring of Fire:
- The Pacific Ring of Fire is one of the most seismically active regions on Earth, encircling the Pacific Ocean. It is characterized by a series of subduction zones, where one tectonic plate is forced beneath another. This convergent plate boundary interaction results in powerful earthquakes, volcanic eruptions, and the formation of deep ocean trenches.
- Countries located along the Ring of Fire, such as Japan, Indonesia, the Philippines, New Zealand, and the west coast of North and South America, frequently experience significant seismic activity.
- Himalayan Seismic Belt:
- The Himalayan region is a major earthquake belt where the Indian plate is colliding with the Eurasian plate. This collision has created the Himalayan mountain range and is responsible for frequent earthquakes in the region.
- Countries like Nepal, India, and parts of Pakistan experience significant seismic activity along this belt.
- San Andreas Fault Zone:
- The San Andreas Fault is a transform plate boundary in California, USA, where the Pacific Plate and the North American Plate slide past each other horizontally. This transform boundary is known for generating large earthquakes.
- Cities like Los Angeles and San Francisco are located near the San Andreas Fault and have experienced notable earthquakes in the past.
- East African Rift Zone:
- The East African Rift is a continental rift zone where the African plate is splitting into two smaller plates: the Nubian plate and the Somali plate. This process results in tensional stress, leading to earthquakes and volcanic activity.
- Countries in the East African Rift region, such as Ethiopia, Kenya, and Tanzania, often experience seismic events.
- Alpide Belt:
- The Alpide Belt is a seismic zone that extends from the Atlantic Ocean through southern Europe and Asia to the Himalayas. It includes the Mediterranean region, the Middle East, and parts of Asia.
- This belt is associated with the convergence of the African plate, Eurasian plate, and Indian plate, leading to earthquakes and mountain-building processes.
- Mid-Atlantic Ridge:
- The Mid-Atlantic Ridge is a divergent plate boundary that runs along the floor of the Atlantic Ocean. It is marked by the upwelling of magma, which creates new oceanic crust and occasionally leads to earthquakes.
- Although the Mid-Atlantic Ridge is underwater, it is a significant earthquake belt, especially in regions where it intersects with continental landmasses.
These earthquake belts are dynamic and are the result of ongoing tectonic processes. The interactions between tectonic plates, including subduction, collision, and lateral sliding, play a central role in the generation of seismic activity in these regions. Understanding earthquake belts is essential for earthquake monitoring, preparedness, and risk assessment in vulnerable areas.
Earthquakes in India
Earthquakes in India are a common natural hazard due to the country’s location in a seismically active region. India is situated at the complex boundary of the Indian Plate and several neighboring tectonic plates, which leads to frequent seismic activity. Here’s an overview of earthquakes in India:
- Tectonic Setting:
- India is positioned on the Indian Plate, which is converging with the Eurasian Plate to the north. This collision has resulted in the uplift of the Himalayan mountain range.
- The Indian Plate is also interacting with the Australian Plate to the south, the Arabian Plate to the west, and the Burmese Plate to the east, leading to various types of tectonic interactions and seismic activity.
- Himalayan Seismic Belt:
- The Himalayan region is one of the most seismically active areas in India. It experiences earthquakes due to the ongoing collision of the Indian Plate with the Eurasian Plate.
- Notable earthquakes in this region have caused significant damage and loss of life, including the 1905 Kangra earthquake and the 1934 Bihar-Nepal earthquake.
- North-Eastern Region:
- The northeastern states of India, including Assam, Arunachal Pradesh, and Manipur, are located in a seismically active region. This area is influenced by the interaction of the Indian Plate with the Burmese Plate.
- The 1950 Assam-Tibet earthquake is one of the largest earthquakes in the region’s history.
- Peninsular India:
- Although less seismically active than the northern and northeastern regions, peninsular India is not immune to earthquakes.
- The Koynanagar earthquake of 1967 in Maharashtra is one of the significant earthquakes in this region.
- Western and Northern Regions:
- The western part of India, particularly Gujarat, has experienced devastating earthquakes. The 2001 Gujarat earthquake is a notable example.
- The northern regions of India, including Jammu and Kashmir and Himachal Pradesh, also witness seismic activity related to the Himalayan convergence.
- Andaman and Nicobar Islands:
- The Andaman and Nicobar Islands, located in the eastern part of the Indian Ocean, are susceptible to earthquakes due to their proximity to the boundary between the Indian Plate and the Burmese Plate.
- Seismic Risk and Preparedness:
- Earthquake risk in India is a significant concern, especially in densely populated areas. Building construction standards and earthquake preparedness measures are critical to reducing the impact of seismic events.
- The National Disaster Management Authority (NDMA) and various state-level agencies are responsible for earthquake preparedness, response, and mitigation efforts.
- Monitoring and Research:
- India has a network of seismological observatories and research institutions dedicated to monitoring and studying earthquakes.
- Efforts are made to improve earthquake prediction, early warning systems, and public awareness.
In summary, India’s geographical location in a seismically active region exposes it to earthquakes of varying magnitudes. The country faces the ongoing challenge of managing earthquake risk, implementing building codes, and enhancing disaster preparedness to reduce the impact of seismic events on its population and infrastructure.
Seismic Zoning of India (Zone 1 to Zone 5)
India is divided into several seismic zones, ranging from Zone 1 to Zone 5, based on the level of seismic activity and the expected intensity of earthquakes in each zone. These seismic zones are used to assess earthquake risk, design construction standards, and implement mitigation measures. Here is an overview of India’s seismic zones:
Zone 1:
- Zone 1 is the least seismically active region in India.
- This zone covers parts of northern and northwestern India, including parts of Gujarat, Rajasthan, and Jammu and Kashmir.
- Earthquakes in this zone are relatively rare, and their intensity is expected to be low.
- Building construction standards in this zone are the least stringent.
Zone 2:
- Zone 2 includes regions with a low to moderate level of seismic activity.
- This zone covers areas in northern India, including Delhi, parts of Uttar Pradesh, Haryana, and Punjab.
- While earthquakes in this zone are less frequent than in some other zones, moderate-intensity earthquakes can occur.
- Building codes in this zone require some seismic-resistant features.
Zone 3:
- Zone 3 is characterized by moderate seismic activity.
- This zone includes parts of northern and northeastern India, such as West Bengal, Bihar, and parts of Uttar Pradesh and Assam.
- Earthquakes in this zone can have a moderate to significant impact.
- Building construction standards are more stringent in Zone 3 to enhance earthquake resistance.
Zone 4:
- Zone 4 is known for a high level of seismic activity.
- This zone covers regions in the Himalayan foothills, parts of Himachal Pradesh, Uttarakhand, and Jammu and Kashmir.
- Earthquakes in this zone can be strong, and there is a higher risk of structural damage.
- Building codes in this zone require robust seismic-resistant construction techniques.
Zone 5:
- Zone 5 is the highest-risk seismic zone in India.
- It includes areas in the northeastern states, parts of Himachal Pradesh, and some portions of Jammu and Kashmir.
- This zone experiences the most significant seismic activity, including the potential for very strong earthquakes.
- Building construction standards in Zone 5 are the strictest to ensure the structural integrity of buildings during powerful earthquakes.
It’s essential to note that seismic zoning plays a critical role in determining building codes, construction practices, and disaster preparedness measures in various regions of India. Structures in higher seismic zones are required to meet more rigorous standards to withstand the forces generated by earthquakes. The goal is to reduce the risk to life and property in the event of seismic events.
Top of Form
Layers of Atmosphere
The Earth’s atmosphere is composed of several layers, each with its own characteristics and significance. These layers play crucial roles in supporting life, influencing weather patterns, and protecting our planet. Let’s explore the layers of the atmosphere from the ground up:
- Troposphere:
- Altitude Range: The troposphere is the layer closest to Earth’s surface and extends from the ground to an altitude of about 8 to 15 kilometers (5 to 9 miles) above sea level, depending on location and season.
- Significance: This is where weather occurs. It contains most of Earth’s clouds and is responsible for the air we breathe. As you go higher in the troposphere, the temperature decreases.
- Stratosphere:
- Altitude Range: The stratosphere is situated above the troposphere and extends from about 15 to 50 kilometers (9 to 31 miles) above sea level.
- Significance: The stratosphere contains the ozone layer, which absorbs and filters out a significant portion of the sun’s harmful ultraviolet (UV) radiation. This layer protects living organisms from excessive UV exposure.
- Mesosphere:
- Altitude Range: The mesosphere extends from approximately 50 to 85 kilometers (31 to 53 miles) above sea level.
- Significance: It’s the layer where meteors and small debris from space burn up upon entry into the atmosphere. Temperatures here decrease with altitude, making it extremely cold.
- Thermosphere:
- Altitude Range: The thermosphere starts around 85 kilometers (53 miles) and extends upward indefinitely.
- Significance: In the thermosphere, temperatures rise significantly due to the absorption of high-energy solar radiation. This layer contains the International Space Station (ISS) and other low Earth orbiting satellites. It also plays a role in radio signal reflection.
- Exosphere:
- Altitude Range: The exosphere is the outermost layer and gradually transitions into space. There’s no well-defined upper limit.
- Significance: This layer marks the boundary between Earth’s atmosphere and outer space. It’s extremely thin and contains very few gas molecules. Satellites in geostationary orbit and beyond reside in the exosphere.
These layers of the atmosphere collectively serve several crucial functions:
- Protection from Harmful Radiation: The ozone layer in the stratosphere shields us from harmful UV radiation, preventing skin damage and other health issues.
- Weather and Climate: The troposphere is where weather phenomena, such as rain, clouds, and storms, take place. It also influences climate patterns on Earth.
- Breathable Air: The troposphere contains the oxygen we breathe, making it essential for life on Earth.
- Orbital Space Operations: The thermosphere and exosphere are where satellites and the ISS orbit. Understanding these layers is crucial for space exploration and satellite communication.
- Protection from Space Debris: The mesosphere acts as a barrier, disintegrating most meteors and debris from space before they reach the Earth’s surface.
Each layer of the atmosphere is interconnected and contributes to the overall stability and habitability of our planet. Understanding their significance helps us appreciate the complex interactions that occur in our atmosphere and their impact on life on Earth.
Troposphere
The troposphere is the lowest layer of Earth’s atmosphere, and it is the layer in which most weather phenomena occur. It extends from the Earth’s surface up to an average altitude of about 8 to 15 kilometers (5 to 9 miles) above sea level, although this altitude can vary with latitude and season.
Here are some key characteristics and functions of the troposphere:
- Temperature Decrease with Altitude: As you ascend through the troposphere, the temperature generally decreases. This temperature decrease occurs because the troposphere is heated from below by the Earth’s surface. On average, the temperature decreases by about 6.5 degrees Celsius per kilometer of altitude, a phenomenon known as the environmental lapse rate.
- Weather and Climate: Virtually all weather phenomena, including clouds, precipitation, storms, and wind patterns, take place in the troposphere. This layer is the most turbulent and dynamic part of the atmosphere and is crucial for Earth’s climate system.
- Composition: The troposphere contains the majority of the atmosphere’s water vapor, as well as gases like nitrogen, oxygen, and trace amounts of other gases. Water vapor plays a significant role in the Earth’s energy balance and weather processes.
- Pressure Gradient: Air pressure decreases with increasing altitude in the troposphere. This decrease in pressure is due to the decreasing density of air molecules as you move upward in the atmosphere.
- Tropopause: The boundary between the troposphere and the layer above it, known as the stratosphere, is called the tropopause. The tropopause is characterized by a stable temperature profile, where the temperature remains relatively constant or may even increase slightly with altitude.
- Jet Streams: The troposphere is home to the polar and subtropical jet streams, which are high-speed, narrow air currents that influence weather patterns and aviation routes. These jet streams are located in the upper troposphere, near the tropopause.
- Human Activity: Human activities, including pollution and the release of greenhouse gases, can affect the troposphere. The accumulation of greenhouse gases in the troposphere contributes to global warming and climate change.
- Altitude and Geography: The altitude of the tropopause varies with geographical location and season. It is generally higher over the equator and lower over the poles. Additionally, mountain ranges can disrupt the troposphere’s uniformity.
In summary, the troposphere is the atmospheric layer closest to the Earth’s surface and is where weather processes and most atmospheric phenomena occur. It plays a crucial role in regulating Earth’s climate and is a dynamic and complex layer of the atmosphere.
Tropopause
The tropopause is a significant boundary in Earth’s atmosphere that separates the troposphere, the lowest layer of the atmosphere, from the layer above known as the stratosphere. It is a transition zone characterized by distinct temperature and atmospheric pressure changes. Here’s an explanation of the tropopause:
- Location and Altitude:
- The tropopause is located above the Earth’s surface and marks the boundary between the troposphere and the stratosphere.
- Its altitude varies depending on geographic location, season, and latitude but typically ranges from approximately 8 to 15 kilometers (5 to 9 miles) above sea level. However, it can be higher or lower in specific conditions.
- Temperature Profile:
- One of the defining features of the tropopause is its unique temperature profile. In the troposphere, temperatures generally decrease with increasing altitude, following a pattern known as the environmental lapse rate.
- However, at the tropopause, this lapse rate changes dramatically. Instead of decreasing, temperatures at the tropopause remain relatively constant or may even increase slightly with altitude. This is due to the presence of the ozone layer in the stratosphere, which absorbs and traps incoming solar radiation, leading to a temperature inversion.
- Stability:
- The tropopause is a stable region of the atmosphere, which means it resists vertical air movement and turbulence. This stability is due to the temperature inversion mentioned earlier, which prevents warm, moist air in the troposphere from rising into the stratosphere.
- Importance:
- The tropopause plays a crucial role in influencing weather patterns and atmospheric processes. Weather systems, including clouds, storms, and precipitation, typically occur in the troposphere below the tropopause.
- It also affects the behavior of jet streams, which are fast-moving, narrow air currents that meander along the upper troposphere and can have a significant impact on weather and aviation routes.
- Variability:
- The altitude of the tropopause is not constant and can vary with geographical location and season. For example, the tropopause is generally higher over the equator and lower over the poles.
- Mountain ranges can disrupt the regular altitude of the tropopause, causing it to fluctuate in response to local topography.
In summary, the tropopause is the boundary between the troposphere and the stratosphere and is characterized by a stable temperature inversion. It has a significant influence on weather patterns, atmospheric stability, and the behavior of jet streams. Understanding the tropopause is essential for meteorology and atmospheric science.
Stratosphere
The stratosphere is the second major layer of Earth’s atmosphere, situated above the troposphere and below the mesosphere. It extends from approximately 15 kilometers (9 miles) to about 50 kilometers (31 miles) above the Earth’s surface. The stratosphere has several distinctive characteristics:
- Temperature Profile:
- One of the defining features of the stratosphere is its unique temperature profile. Unlike the troposphere below it, where temperatures generally decrease with increasing altitude, the stratosphere exhibits a temperature inversion. This means that temperatures in the stratosphere tend to increase with altitude, primarily due to the presence of ozone (O3) molecules.
- The increase in temperature within the stratosphere is due to the absorption of ultraviolet (UV) radiation from the sun by ozone molecules. This absorption of UV radiation creates a stable layer that prevents the mixing of air masses between the troposphere and the stratosphere.
- Ozone Layer:
- The stratosphere is home to the ozone layer, which is a region with a relatively high concentration of ozone molecules. The ozone layer is crucial for life on Earth because it absorbs and scatters a significant portion of the sun’s harmful ultraviolet (UV) radiation.
- The ozone layer forms a protective shield that shields the Earth’s surface from the harmful effects of excessive UV radiation, which can cause skin cancer, cataracts, and harm to ecosystems.
- Airplane Altitudes:
- Commercial airliners typically cruise in the lower part of the stratosphere, known as the lower stratosphere. Flying at these altitudes allows aircraft to avoid most of the weather disturbances and turbulence present in the troposphere, resulting in smoother flights.
- Lack of Weather Systems:
- The stratosphere is characterized by a lack of weather systems, such as clouds, storms, and precipitation, that are common in the troposphere. This stability is due to the temperature inversion and the absence of vertical mixing of air masses.
- Jet Streams:
- High-altitude jet streams, such as the polar and subtropical jet streams, are located in the stratosphere, near the boundary with the troposphere. These fast-moving air currents play a significant role in influencing weather patterns and are important for aviation routes.
- Human Impact:
- Human activities, such as the release of chlorofluorocarbons (CFCs), have had a detrimental effect on the ozone layer in the stratosphere. The depletion of ozone in the ozone layer, especially over polar regions, has led to the formation of the ozone hole, which poses environmental and health risks.
In summary, the stratosphere is the atmospheric layer above the troposphere and is characterized by a temperature inversion, the presence of the ozone layer, and a lack of typical weather systems. It plays a crucial role in protecting life on Earth from harmful UV radiation and is important for aviation and atmospheric science.
How temperature varies with height in Atmosphere?
The variation of temperature with height in Earth’s atmosphere is not uniform and can be divided into different layers, each with its own temperature characteristics. This variation is important for understanding atmospheric dynamics and the behavior of weather systems. Here’s how temperature generally varies with height in the atmosphere:
- Troposphere:
- The troposphere is the lowest layer of the atmosphere, extending from the Earth’s surface to an average altitude of about 8 to 15 kilometers (5 to 9 miles) depending on location and season.
- In the troposphere, temperatures typically decrease with increasing altitude. On average, the temperature decreases at a rate of about 6.5 degrees Celsius per kilometer (3.5 degrees Fahrenheit per 1,000 feet). This decrease is known as the environmental lapse rate.
- This cooling trend occurs because the troposphere is primarily heated from below by the Earth’s surface. As you ascend, you move away from the heat source, causing temperatures to drop.
- Tropopause:
- The tropopause is the boundary between the troposphere and the stratosphere, and it is characterized by a stable temperature profile. Temperatures at the tropopause remain relatively constant or may even increase slightly with altitude.
- The stability of the tropopause is due to the presence of the ozone layer in the stratosphere, which absorbs and traps incoming solar radiation, causing a temperature inversion.
- Stratosphere:
- The stratosphere is situated above the tropopause and extends to approximately 50 kilometers (31 miles) above the Earth’s surface.
- In the stratosphere, temperatures generally increase with altitude, primarily because of the presence of ozone (O3) molecules. Ozone absorbs ultraviolet (UV) radiation from the sun, warming the stratosphere.
- This temperature increase within the stratosphere is in contrast to the troposphere, where temperatures decrease with altitude.
- Mesosphere and Thermosphere:
- The mesosphere, situated above the stratosphere, experiences a temperature decrease with altitude similar to the troposphere.
- The thermosphere, located even higher in the atmosphere, exhibits a temperature increase with altitude due to the absorption of high-energy solar radiation by the sparse gas molecules present.
- Exosphere:
- The exosphere, the outermost layer of the atmosphere, is characterized by extremely low densities and high temperatures due to solar radiation. However, these temperatures are not representative of the conditions experienced by spacecraft and satellites, as the exosphere’s particles are so sparse that they have little thermal impact.
In summary, the temperature variation with height in the atmosphere is not constant but varies by atmospheric layer. The troposphere, stratosphere, mesosphere, and thermosphere each exhibit distinct temperature characteristics, and the presence of specific gases, particularly ozone, plays a crucial role in determining these temperature patterns. Understanding these variations is fundamental to meteorology and atmospheric science.
Temperature Inversion
A temperature inversion is a meteorological phenomenon in which the usual vertical temperature profile of the atmosphere is reversed or inverted. Instead of temperatures decreasing with increasing altitude, as is the norm, temperature inversions involve a layer of air in which temperatures increase with altitude. Temperature inversions are essential atmospheric phenomena with various implications for weather, air quality, and aviation. Here’s how temperature inversions work:
Key Characteristics of Temperature Inversions:
- Stable Air: Temperature inversions are associated with stable atmospheric conditions. In stable air, parcels of air tend to resist vertical mixing, leading to the development of distinct layers with different temperature characteristics.
- Nocturnal Inversions: Temperature inversions often occur during the nighttime or early morning hours, particularly on clear and calm nights. This is because the Earth’s surface loses heat rapidly through radiation, cooling the air near the ground.
- Clear Skies: Clear skies are conducive to the radiational cooling of the Earth’s surface, which can result in temperature inversions. Cloud cover acts as a thermal blanket, trapping heat and preventing the surface from cooling rapidly.
Causes of Temperature Inversions:
- Radiational Cooling: The primary cause of temperature inversions is radiational cooling of the Earth’s surface. After sunset, the ground loses heat through radiation, causing the air near the surface to cool rapidly. This cooler air forms a layer near the ground.
- Warm Air Aloft: Above the cooling layer near the surface, a layer of warmer air may be present. This warm air aloft can act as a lid or cap, preventing the cooler air near the surface from mixing with the warmer air above. This creates the inversion layer.
Implications of Temperature Inversions:
- Air Quality: Temperature inversions can trap pollutants near the surface, leading to poor air quality. Inversions prevent the dispersion of pollutants into the upper atmosphere, which can have adverse health effects for those living in affected areas.
- Fog and Low Clouds: Inversions can lead to the formation of fog and low-level clouds, especially in valleys and near bodies of water. Moisture in the cooler air near the surface condenses, leading to reduced visibility.
- Temperature Contrast: Inversions can result in significant temperature differences between the surface layer and the layer above, which can affect agriculture, frost formation, and temperature-sensitive vegetation.
- Aviation: Temperature inversions can lead to turbulence and other aviation challenges. They can also affect the performance of aircraft and create visibility issues, particularly in valleys.
In summary, a temperature inversion is a reversal of the typical temperature profile in the atmosphere, where temperatures increase with altitude instead of decreasing. These inversions are often associated with stable atmospheric conditions, radiational cooling, and a warm layer of air aloft. They have various effects on weather, air quality, and aviation and are a critical consideration in meteorology and atmospheric science.
Ozone Layer
The ozone layer is a region of Earth’s stratosphere that contains a relatively high concentration of ozone (O3) molecules. It is located approximately 10 to 50 kilometers (6 to 31 miles) above the Earth’s surface, within the stratosphere. The ozone layer is characterized by its importance in protecting life on Earth from the harmful effects of ultraviolet (UV) radiation from the sun. Here’s a detailed explanation of the ozone layer:
- Composition:
- The ozone layer consists of ozone molecules, which are composed of three oxygen atoms (O3). In contrast, the majority of the Earth’s atmosphere is composed of diatomic oxygen molecules (O2), consisting of two oxygen atoms.
- Formation:
- Ozone in the stratosphere is primarily formed through the absorption of high-energy, short-wavelength UV radiation from the sun. This energy causes the dissociation (splitting) of diatomic oxygen molecules (O2) into individual oxygen atoms (O), which can then react with other diatomic oxygen molecules to form ozone (O3).
- The overall process can be summarized as follows:
- UV radiation splits O2 into two oxygen atoms: O2 + UV → 2O
- Oxygen atoms (O) react with diatomic oxygen (O2) to form ozone (O3): O + O2 → O3
- Ozone Distribution:
- The ozone layer is not evenly distributed throughout the stratosphere but is concentrated in a specific region. Most of the ozone is found between 15 and 35 kilometers (9 to 22 miles) above the Earth’s surface.
- Ozone’s Protective Role:
- The ozone layer plays a vital role in protecting life on Earth by absorbing and blocking a significant portion of the sun’s harmful UV radiation. Specifically, it absorbs the highly energetic UV-C and most UV-B radiation.
- Without the ozone layer, these harmful UV rays would reach the Earth’s surface in much greater quantities, leading to adverse effects on human health, including increased rates of skin cancer, cataracts, and sunburn, as well as damage to terrestrial and aquatic ecosystems.
- Ozone Depletion:
- Human activities, such as the release of chlorofluorocarbons (CFCs) and other ozone-depleting substances, have led to a thinning of the ozone layer, especially over polar regions. This phenomenon is known as ozone depletion.
- The most significant ozone hole is observed over Antarctica during the Southern Hemisphere’s spring, and it is caused by a complex interplay of chemical reactions involving ozone-depleting substances.
- International agreements, such as the Montreal Protocol, have successfully led to the reduction of ozone-depleting substances and efforts to recover the ozone layer.
In summary, the ozone layer is a region in the stratosphere containing ozone molecules that protect life on Earth by absorbing and blocking harmful UV radiation from the sun. Its formation and distribution are essential for maintaining a habitable environment on our planet, and it serves as a critical shield against the adverse effects of excessive UV radiation.
Mesosphere
The mesosphere is a layer of Earth’s atmosphere located above the stratosphere and below the thermosphere. It extends from an altitude of approximately 50 kilometers (31 miles) to about 85 kilometers (53 miles) above the Earth’s surface. The mesosphere is characterized by unique features and conditions:
- Temperature Profile:
- In the mesosphere, temperatures generally decrease with increasing altitude, similar to the troposphere, which is the lowest atmospheric layer. This cooling trend is due to the decreasing density of air molecules with height and the absence of significant heating mechanisms.
- Mesopause:
- The boundary between the mesosphere and the thermosphere, called the mesopause, is characterized by the coldest temperatures in Earth’s atmosphere. At this altitude, temperatures can drop to as low as -90 degrees Celsius (-130 degrees Fahrenheit).
- Meteors and Meteoroids:
- The mesosphere is the region where most meteors and meteoroids burn up upon entry into Earth’s atmosphere. These small rocky or metallic objects from space are known as meteoroids when they are outside the atmosphere but become meteors (shooting stars) as they burn and vaporize in the mesosphere due to the intense friction and heat generated during their high-speed entry.
- Noctilucent Clouds:
- Noctilucent clouds, also called polar mesospheric clouds, are thin and wispy clouds that can be observed in the mesosphere, primarily at high latitudes near the polar regions. These clouds are made of ice crystals and can only form at extremely low temperatures in the upper mesosphere.
- Limited Human Activity:
- The mesosphere is well above the altitudes at which aircraft typically operate, and it is too low for spacecraft orbits. As a result, there is limited human activity in this layer, and it is challenging to study directly.
- Ionization:
- In the upper mesosphere, some gases become ionized by solar radiation. This ionization can affect radio wave propagation and communications but to a lesser extent than the more ionized thermosphere above.
- Extreme Cold and Low Pressure:
- The mesosphere is an extremely cold and low-pressure environment. Because of its high altitude and lack of significant heating mechanisms, it is one of the coldest regions in Earth’s atmosphere.
- Transition Layer:
- The mesosphere serves as a transitional layer between the denser and more turbulent layers below (the troposphere and stratosphere) and the thinner, less dense layers above (the thermosphere and exosphere).
In summary, the mesosphere is a distinct layer of Earth’s atmosphere situated above the stratosphere and characterized by decreasing temperatures with height. It is known for its role in meteor and meteoroid interactions, the formation of noctilucent clouds, and its extreme cold temperatures. While it has limited human activity, it plays a crucial role in Earth’s atmospheric dynamics and interactions with space objects.
Solar radiation and Insolation
Solar radiation, often referred to as insolation, is the energy emitted by the Sun in the form of electromagnetic waves, including visible light, ultraviolet (UV) radiation, and infrared radiation. This energy is the primary source of heat and light for Earth and is essential for maintaining the planet’s climate, supporting life, and driving various Earth processes. Here’s an explanation of solar radiation and insolation:
- Solar Radiation Composition:
- Solar radiation consists of a spectrum of electromagnetic waves emitted by the Sun. These waves include:
- Visible Light: The Sun emits a significant portion of its energy as visible light, which is the range of light that can be seen by the human eye.
- Ultraviolet (UV) Radiation: UV radiation is a higher-energy form of light that is not visible to the human eye. It includes UVA, UVB, and UVC rays, with varying degrees of energy and effects on living organisms.
- Infrared (IR) Radiation: Infrared radiation consists of longer-wavelength waves beyond the red end of the visible light spectrum. It includes near-infrared, mid-infrared, and far-infrared radiation.
- Transmission through Space:
- Solar radiation travels through the vacuum of space and reaches Earth without the need for a medium. It travels as electromagnetic waves at the speed of light (about 299,792 kilometers per second or 186,282 miles per second).
- Absorption and Reflection:
- Upon reaching the Earth’s atmosphere, solar radiation can be absorbed, scattered, or reflected. Some of it is absorbed by atmospheric gases and aerosols, while clouds and other particles scatter and reflect some of the radiation back into space.
- Insolation:
- The term “insolation” is derived from “incoming solar radiation.” It specifically refers to the amount of solar radiation received at a particular location on Earth’s surface. Insolation is measured in units such as watts per square meter (W/m²).
- Insolation depends on factors such as the time of day, latitude, season, and local atmospheric conditions. It varies throughout the day and year due to the tilt of Earth’s axis and its orbit around the Sun.
- Energy Transfer and Climate:
- Solar radiation plays a critical role in Earth’s climate. It heats the Earth’s surface, causing it to warm. This warmth is then transferred through various atmospheric and oceanic processes, influencing weather patterns, ocean currents, and climate zones.
- Solar Energy Utilization:
- Solar radiation is harnessed for various practical purposes, including solar power generation (solar panels), solar water heating, and photosynthesis in plants. Solar energy is considered a clean and renewable energy source.
- Impact on Life:
- Solar radiation is essential for life on Earth. It drives photosynthesis in plants, which forms the basis of the food chain. However, excessive exposure to UV radiation can be harmful to living organisms and can cause skin damage and increase the risk of skin cancer.
In summary, solar radiation, also known as insolation, is the energy emitted by the Sun in the form of electromagnetic waves. It plays a fundamental role in Earth’s climate, sustains life through photosynthesis, and has practical applications in energy production. Understanding solar radiation is crucial for studying weather patterns, climate, and sustainable energy sources.
Exosphere
The exosphere is the outermost layer of Earth’s atmosphere, extending from the upper boundary of the thermosphere to the edge of space. While it is part of Earth’s atmosphere, the exosphere is unlike the layers below it in several significant ways:
- Extreme Altitude: The exosphere begins at an altitude of about 500 kilometers (310 miles) above the Earth’s surface and extends to the exobase, which is often considered to be around 10,000 kilometers (6,200 miles) or more. This makes the exosphere the highest atmospheric layer.
- Transition to Space: The exosphere represents the transition between Earth’s atmosphere and outer space. In this region, the density of air molecules is exceedingly low, and there is no distinct boundary that separates it from the vacuum of space. Instead, the exosphere gradually merges with the interplanetary medium.
- Minimal Air Density: The exosphere has an extremely low air density, with air molecules being few and far between. As a result, it can be described as almost a vacuum. This low density is due to the decreasing number of air molecules as altitude increases.
- Gas Molecules: Although the exosphere is sparsely populated with gas molecules, it still contains trace amounts of various gases, including hydrogen (H2), helium (He), oxygen (O2), and other light gases. These gases are present in very small quantities.
- Lack of Thermal Conductivity: Unlike the lower layers of the atmosphere, the exosphere lacks the thermal c